Assistant Professor, Department of Mechanical and Mechatronics Engineering
- FMA
- The Fabricator
- FABTECH
- Canadian Metalworking
Next-generation welding tech
A survey of some of the most important topics in welding research and industry today
- By Adrian Gerlich
- December 20, 2018
- Article
- Welding
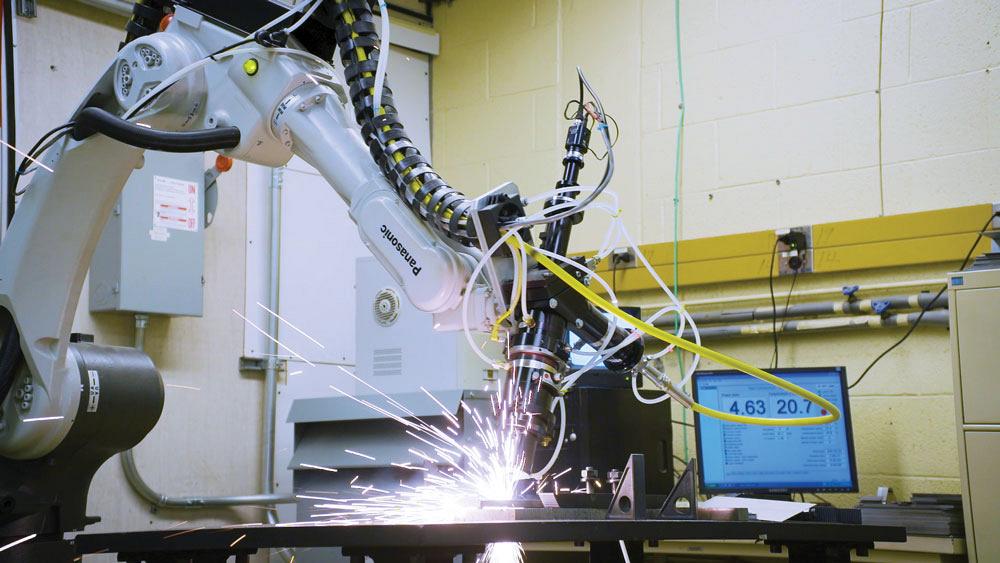
In the lab, laser welding power sources have been reported at up to 100 kW, which can weld more than 50 mm of steel in a single pass. In this photo we wee a high-speed fibre laser welding ultrahigh-strength steels for automotive applications.
Most experts would consider welding to be a mature technology. Yet during the past two decades, considerable advances have been made in developing new technology for joining new high-strength materials, integrating intelligent automation, and applying various welding processes to additive manufacturing (AM) of components. Surveying the trends in research and industrial sectors provides some perspective on where there may be growing knowledge gaps for innovation, and potential skills gaps for future welders and engineers.
Hot Topics
In 2012 the International Institute of Welding (IIW) conducted a survey of more than 60 experts around the world to identify the innovations that were making the greatest impact in welding [1]. It addressed ongoing challenges related to new materials and their weldability. Some of the new technologies indicated as growth areas were robotic, electron beam, laser, hybrid laser, friction stir, and magnetic pulse welding. The published literature since 2013 until 2018 suggests that these continue to be hot-button topics in scientific research. For example, during this period, of the top 10 most cited papers that show “welding” as a topic [2-11] all involve laser, electron beam, or friction stir processes; however, eight of these papers apply these processes to AM or 3-D printing of metal components. This is not terribly surprising, as there has been an astonishing rate of investment both in the public and private sector directed toward AM research to enable printing of large metal components and structures.
Although commercial systems based on laser heating of a powder bed are used in a range of industries, wire-arc AM [12] is only starting to gain acceptance in manufacturing. One of the most notable applications of the process is the pedestrian bridge fabricated by MX3D [13] using robotic gas metal arc welding. Although this example is an impressive one-off showpiece, this technology is being recognized as an economically viable one for other large components such as naval ship propellers; in fact, a 1.35-m-diameter propeller with a mass of 400 kg has been 3-D printed [14]. It is expected that other applications will come to market, requiring skilled workers with the ability to combine welding knowledge with robotic automation and feedback controls. More advanced systems use electron beam heat sources to deposit metal with closed-loop geometry control [15], and some of these are now being installed at AM service providers in Canada [16].
Recently friction stir welding has been adapted to facilitate deposition of material for AM [17]; this offers some unique advantages in terms of build rate and material properties. Friction stir welding has been commercially adopted in the automotive industry by Honda and Mazda for applications such as dissimilar aluminum to steel joining [18]. The application of friction stir welding is likely to expand in the automotive sector for construction of electric vehicles [19], where welding of the battery tray usually involves several extruded and formed aluminum components that must be hermetically sealed during welding.
One of the most exotic materials recently joined by friction stir welding is CoCrFeNiMn, which is a high-entropy alloy that can achieve strengths of more than 600 MPa along with strains to fracture exceeding 60 per cent [20]. These high-entropy alloys have attracted a considerable amount of attention in the last several years due to their combination of properties and potential to replace high-grade steels and titanium alloys in aerospace applications or in energy applications where hard coating materials are needed.
Pushing Laser Welding’s Reach
In terms of more traditional joining applications, manufacturing technology advances have continued to bring the cost of high-power laser sources down, while also increasing their quality. In the lab, laser welding power sources have been reported at up to 100 kW, which can weld more than 50 mm of steel in a single pass [21]. However, it becomes progressively more difficult to achieve high penetration depths with such high-power laser sources. One can typically achieve approximately 1 mm of penetration per kilowatt, while above 20 kW this decreases to about 0.5 mm per kilowatt because of losses in energy efficiency. Control of the penetration for such high-power lasers is critical, and technology has developed a high-speed feedback system to monitor and control the penetration depth of a laser keyhole during welding [22].
To weld thick sections with lower powers, techniques such as hybrid welding [23] and laser scanning or high-speed oscillation [24] have been demonstrated as approaches to decrease the required power for many specialized applications. The use of hybrid laser-arc welding has increased the capability of laser welding for thicker materials, but the complexity of the process increases considerably due to the added variables, such as wire feed speed and arc current, arc standoff distance, torch angle, and shielding gas selection [25].
The benefits of hybrid laser-arc welding to reduce heat input and decrease thermal distortion have been realized in the shipbuilding industry [26], and this has led to the deployment of this technology in many shipyards across Europe over the past two decades. This is a logical niche application, since joining the structures in naval applications typically consist of large panels which are prone to distortion, and correcting these distortions has traditionally accounted for a significant percentage of manual labour costs.
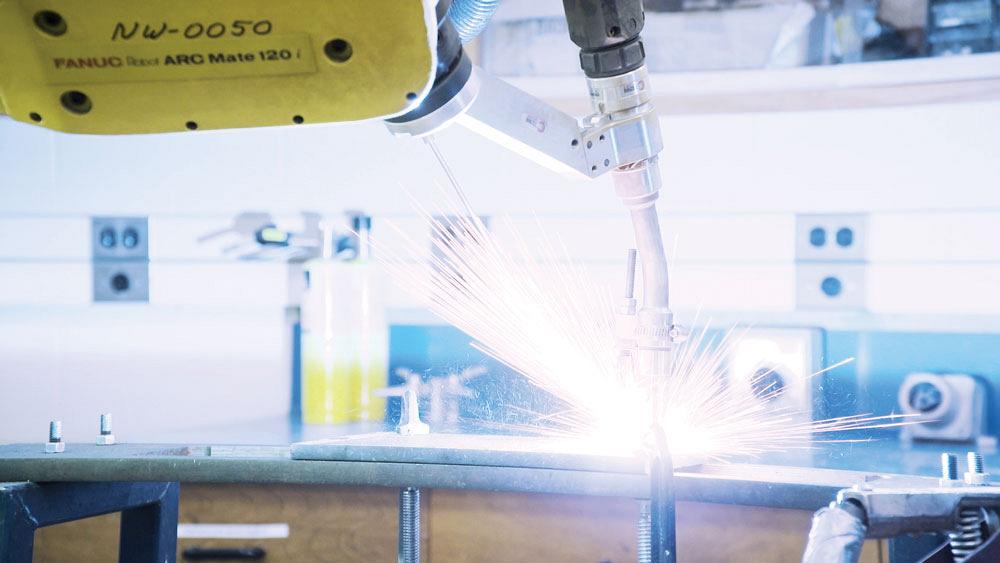
Here hybrid gas metal arc welding using cold wire additions increases metal deposition rate and changes weld metal chemistry in real time.
Reduced distortion is also a major requirement for the automotive industry, and this has made hybrid laser-arc welding a popular choice for joining high-strength thin sheets in automotive body structures and brazing roof panels. The use of lasers in automotive dates back to the 1976 Ford Torino, on which CO2 lasers were used to join body panels; however, large-scale production did not occur until the use of laser welding for the roof in the BMW 3-series in 1985 [27]. The automotive industry has widely adopted remote laser welding since 2007 [28], in which the laser is scanned much faster than the travel of the robot arm. This technology has begun to displace traditional resistance spot welding methods for situations in which higher production rates are required [29], since a small circular or C-shaped laser weld can provide comparable strength. These scanning strategies have also been used in laser seam welding, in which oscillating in a circular or weaving profile can reduce defects in aluminum joining or facilitate dissimilar joining of materials [30].
Considering many of the steels in automotive now range in strength from 980 to 1,500 MPa, the high energy and fast welding speeds offered by lasers can avoid the deterioration of the heat-affected zone in these materials. However, in other industries that use thicker-gauge materials, avoiding this softening while meeting toughness requirements in new high-strength steels with yield points over 1,200 MPa remains a challenge [31]. There has been a considerable push toward the application of both autogenous lasers and laser hot-wire-assisted processes for large-scale structural applications in Japan, with deployment of these methods for bridge deck panels since 2014 [32]. These applications have considerable fatigue demands, and laser hot-wire-assisted welding offers new abilities to control the geometry of the fillet toe geometry [33], which is a major advantage since this shape has a direct influence on fatigue crack initiation.
Real-time Feedback and Monitoring
Other cost reductions in robotics, sensing, and signal processing have enabled features such automated seam tracking (either through the arc or using an external laser) in automation for more than two decades [34, 35]. The miniaturization of sensors and processing power has enabled new capabilities, such as training systems that use augmented reality [36] and real-time monitoring to provide welders feedback on their travel speed or potential defect formation [37].
Some recent advances in thermal cameras also have driven down the cost of sensors, while also increasing their robustness for industrial applications. Commercial systems have been developed that enable both thermal monitoring [38, 39] and even adaptive control of welding using thermal feedback based on pyrometer sensing technology [40, 41]. In parallel with thermal imaging, advances in high-dynamic-range imaging for traditional image monitoring of the weld have enabled much more reliable and clear observations of the welding process and allow one to monitor challenging areas operators are not able to access directly. [42].
With all of these innovations in sensing, data processing and storage become a challenge. Storage becomes important for monitoring applications; this is where the opportunities for quality control become quite attractive. For example, in the case of monitoring thermal signatures, one may co-ordinate non-destructive testing equipment used later in production to inspect areas only where the thermal profile strayed from a set-point. With combined vision, thermal, and electrical signal monitoring, new capabilities will emerge for detecting defects, as all of the systems involved become progressively more interconnected as we embark on the Industry 4.0 revolution.
However, don’t expect all manual welding to become obsolete; we are still far from being able to replace most non-repetitive welding operations with automation. These advancements in sensing can still have an impact in this area, however. For example, a miniaturized spectrometer on a welder’s helmet may indicate that the composition of the weld metal or shielding gas has changed. That signal may be relayed to them through an augmented reality display that is monitoring their torch position [43]. There is clearly a substantial field of innovation opened up by new welding technologies currently being developed or already deployed in industry.
Adrian Gerlich is an associate professor in the Department of Mechanical and Mechatronics Engineering at University of Waterloo, (agerlich@uwaterloo.ca).
References:
- C. Smallbone, M. Kocak, The IIW White Paper, “Improving global quality of life through optimum use and innovation of welding and joining technologies”, International Institute of Welding, 2014
- Carter, Luke N.; Martin, Christopher; Withers, Philip J.; Attallah, Moataz M., (2014) The influence of the laser scan strategy on grain structure and cracking behaviour in SLM powder-bed fabricated nickel superalloy, Journal of Alloys and Compounds, 615, 338-347
- Dehoff, R. R.; Kirka, M. M.; Sames, W. J.; Bilheux, H.; Tremsin, A. S.; Lowe, L. E.; Babu, S. S., (2015) Site specific control of crystallographic grain orientation through electron beam additive manufacturing, Materials Science and Technology, 31(8), 931-938
- Liu, Y. J.; Li, S. J.; Wang, H. L.; Hou, W. T.; Hao, Y. L.; Yang, R.; Sercombe, T. B.; Zhang, L. C., (2016) Microstructure, defects and mechanical behavior of beta-type titanium porous structures manufactured by electron beam melting and selective laser melting, Acta Materialia, 113, 56-67
- Williams, S. W.; Martina, F.; Addison, A. C.; Ding, J.; Pardal, G.; Colegrove, P. (2016) Wire plus Arc Additive Manufacturing, Materials Science and Technology, 32(7), 641-647
- Wang, Zhuqing; Palmer, Todd A.; Beese, Allison M., (2016) Effect of processing parameters on microstructure and tensile properties of austenitic stainless steel 304L made by directed energy deposition additive manufacturing, Acta Materialia, 110, 226-235
- Xin, Renlong; Guo, Changfa; Xu, Zeren; Liu, Guodong; Huang, Xiaoxu; Liu, Qing, (2014) Characteristics of long {10-12} twin bands in sheet rolling of a magnesium alloy, Scripta Materialia, 74, 96-99
- Nie, Pulin; Ojo, O. A.; Li, Zhuguo, (2014) Numerical modeling of microstructure evolution during laser additive manufacturing of a nickel-based superalloy, Acta Materialia, 77, 85-95
- Raghavan, Narendran; Dehoff, Ryan; Pannala, Sreekanth; Simunovic, Srdjan; Kirka, Michael; Turner, John; Carlson, Neil; Babu, Sudarsanam S., (2016) Numerical modeling of heat-transfer and the influence of process parameters on tailoring the grain morphology of IN718 in electron beam additive manufacturing, Acta Materialia, 112, 303-314
- Sahraeinejad, S.; Izadi, H.; Haghshenas, M.; Gerlich, A. P., (2015) Fabrication of metal matrix composites by friction stir processing with different Particles and processing parameters, Materials Science and Engineering A, 626, 505-513
- Ipekoglu, Guven; Cam, Gurel (2014) Effects of Initial Temper Condition and Postweld Heat Treatment on the Properties of Dissimilar Friction-Stir-Welded Joints between AA7075 and AA6061 Aluminum Alloys, Metallurgical and Materials Transactions A, 45A(7), 3074-3087
- http://www.norsktitanium.no/en/News/∼/media/NorskTitanium/Titanidum%20day%20presentations/Paul%20Colegrove%20Cranfield%20Additive%20manufacturing.ashx
- https://mx3d.com/
- https://www.valkwelding.com/en/news/first-3d-printed-ship-propeller
- http://www.sciaky.com/
- https://burloaktech.com/
- http://meldmanufacturing.com/additive-manufacturing/
- https://www.caranddriver.com/features/two-metals-enter-one-metal-leaves-the-miracle-of-friction-stir-welding-tech-dept
- https://electrek.co/2016/02/24/apple-alloy-expert-tesla-spacex/?preview_id=11254
- Shaysultanov, D., Stepanov, N., Malopheyev, S., Vysotskiy, I., Sanin, V., Mironov, S., ... & Zherebtsov, S. (2018). Friction stir welding of a сarbon-doped CoCrFeNiMn high-entropy alloy. Materials Characterization, 145, 353-361.
- Nielsen, S. E. (2015). High power laser hybrid welding–challenges and perspectives. Physics Procedia, 78, 24-34.
- http://www.laserdepth.com/
- Wahba, M., Mizutani, M., & Katayama, S. (2016). Single pass hybrid laser-arc welding of 25 mm thick square groove butt joints. Materials & Design, 97, 1-6.
- Eakkachai, W., Koei, H., Motomichi, Y., Kenji, S., Kota, K., Tadakazu, T., ... & Syoko, T. (2015). Welding Phenomena during Vertical Welding on Thick Steel Plate using Hot-wire Laser Welding Method, Proc. Of the Japanese Welding Society, 33(2), 143s-147s.
- Atabaki, M. M., Yazdian, N., & Kovacevic, R. (2018). Hybrid laser/arc welding of thick high-strength steel in different configurations. Advances in Manufacturing, 6(2), 176-188.
- Kristensen, J. K. (2009). State of art in shipbuilding applications of hybrid laser-arc welding. Proceedings of the NOLAMP12, 1-13.
- https://www.industrial-lasers.com/articles/print/volume-30/issue-3/departments/my-view/five-decades-of-laser-light-in-automotive.html
- https://www.industrial-lasers.com/articles/print/volume-26/issue-5/features/remote-laser-welding-in-automotive-production.html
- https://rts.i-car.com/collision-repair-news/new-welding-methods-for-toyota-lexus.html
- https://www.ipgphotonics.com/en/products/beam-delivery/process-heads/welding/d50-wobble-and-seam-tracking-head
- Kurc-Lisiecka, A., Piwnik, J., & Lisiecki, A. (2017). Laser welding of new grade of advanced high strength steel STRENX 1100 MC. Archives of Metallurgy and Materials, 62(3), 1651-1657.
- https://www.firjan.org.br/lumis/portal/file/fileDownload.jsp?fileId=2C908CEC48AE0F120148E6D6409E3F4A
- Kadoi, K., Shinozaki, K., Yamamoto, M., Owaki, K., Inose, K., & Takayanagi, D. (2011). Development of high-efficiency/high-quality hot-wire laser fillet welding process. Quarterly journal of the Japan Welding Society, 29(3), 62s-65s.
- Jianming Tao and P. Levick, "Assessment of feedback variables for through the arc seam tracking in robotic gas metal arc welding," Proceedings 1999 IEEE International Conference on Robotics and Automation (Cat. No.99CH36288C), Detroit, MI, USA, 1999, pp. 3050-3052 vol.4.
- https://www.robotics.org/filesDownload.cfm?dl=Scherler.PDF
- https://www.millerwelds.com/about/news-releases/new-augmented-reality-welding-system-from-miller-delivers-real-time-feedback
- N. Huang, S. Chen and Y. Zhang, "Machine assisted manual torch operation in gas tungsten arc welding process," 2015 IEEE International Conference on Advanced Intelligent Mechatronics (AIM), Busan, 2015, pp. 1478-1483.
- https://www.sensortherm.de/en/laserapplication
- https://hks-prozesstechnik.de/en/automated-welding/
- T. Springer and A. Welch, (1993) Temperature control during laser vessel welding, Appl. Opt. 32, 517-525
- Papacharalampopoulosa, A., Stavropoulosa, P., & Stavridisa, J. (2017). Adaptive Control of Thermal Processes: Laser Welding and Additive Manufacturing Paradigms.
- https://www.roadtovr.com/augmented-reality-welding-mask/
About the Author
subscribe now
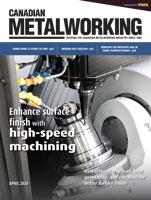
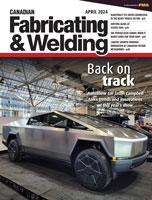
Keep up to date with the latest news, events, and technology for all things metal from our pair of monthly magazines written specifically for Canadian manufacturers!
Start Your Free Subscription- Trending Articles
Aluminum MIG welding wire upgraded with a proprietary and patented surface treatment technology
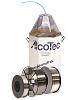
CWB Group launches full-cycle assessment and training program
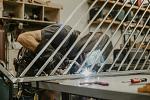
Achieving success with mechanized plasma cutting
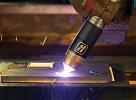
Hypertherm Associates partners with Rapyuta Robotics
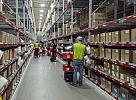
Brushless copper tubing cutter adjusts to ODs up to 2-1/8 in.
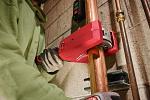
- Industry Events
MME Winnipeg
- April 30, 2024
- Winnipeg, ON Canada
CTMA Economic Uncertainty: Helping You Navigate Windsor Seminar
- April 30, 2024
- Windsor, ON Canada
CTMA Economic Uncertainty: Helping You Navigate Kitchener Seminar
- May 2, 2024
- Kitchener, ON Canada
Automate 2024
- May 6 - 9, 2024
- Chicago, IL
ANCA Open House
- May 7 - 8, 2024
- Wixom, MI